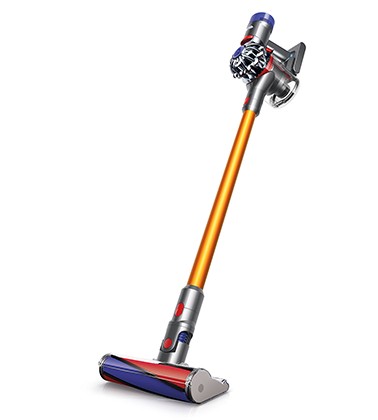
This post is devoted to computations for beta ensembles from random matrix theory.
Real case. Following MR2813333, or MR2325917 MR1936554 with a different scaling, for all $\beta>0$ and $n\geq2$, the $\beta$ Hermite ensemble is the probability measure on $\mathbb{R}^n$ defined by $$
\mathrm{d}P_{\beta,n}(x)
=\frac{\mathrm{e}^{-n\frac{\beta}{4}(x_1^2+\cdots+x_n^2)}}{C_{\beta,n}}\prod_{i<j}|x_i-x_j|^\beta\mathrm{d}x.
$$ Let $X_n=(X_{n,1},\ldots,X_{n,n})\sim P_{\beta,n}$. The normalization $C_{\beta,n}$ can be explicitly computed in terms of Gamma functions via a Selberg integral. Following MR1936554, the law $P_{\beta,n}$ is the distribution of the ordered eigenvalues of the random tridiagonal symmetric $n\times n$ matrix
$$
M_{\beta,n}=\frac{1}{\sqrt{\beta n}}
\begin{pmatrix}
\mathcal{N}(0, 2) & \chi_{(n-1) \beta} & & &\\
\chi_{(n-1) \beta} & \mathcal{N}(0, 2) & \chi_{(n-2) \beta} & & \\
& \ddots & \ddots & \ddots & \\
& & \chi_{2\beta} & \mathcal{N}(0,2) & \chi_{\beta} \\
& & & \chi_{\beta} & \mathcal{N}(0,2)
\end{pmatrix}
$$
where, up to the scaling prefactor $1/\sqrt{\beta n}$, the entries in the upper triangle including the diagonal are independent, follow a Gaussian law $\mathcal{N}(0,2)$ on the diagonal, and $\chi$-laws just above the diagonal with a decreasing parameter with step $\beta$ from $(n-1)\beta$ to $\beta$. In particular $$X_{n,1}+\cdots+X_{n,n}=\mathrm{Trace}(M_{\beta,n})\sim\mathcal{N}\left(0,\frac{2}{\beta}\right).$$
Using standard algebra on Gamma distributions we also get
$$
X_{n,1}^2+\cdots+X_{n,n}^2
=\mathrm{Tr}(M_{\beta,n}^2)
\sim\mathrm{Gamma}\left(\frac{n}{2}+\frac{\beta n(n-1)}{4},\frac{\beta n}{4}\right).
$$ In particular, we have $$\mathbb{E}((X_{n,1}+\cdots+X_{n,n})^2)=\frac{2}{\beta}\quad\text{and}\quad\mathbb{E}(X_{n,1}^2+\cdots+X_{n,n}^2)=\frac{2}{\beta}+n-1.$$
The mean and covariance of the random vector $X_n$ are given, for all $1\leq i\neq j\leq n$, by
$$
\int x_i \, \mathrm{d}P_{\beta,n} =0, \quad
\int x_i^2 \, \mathrm{d}P_{\beta,n} = \frac{n-1}{n} + \frac{2}{n\beta},
\quad \int x_ix_j \, \mathrm{d}P_{\beta,n} = – \frac{1}{n} .
$$
Dynamics. It is also possible to compute using the overdamped Langevin dynamics associated to the Boltzmann-Gibbs measure $P_{\beta,n}$. This is known as the Dyson-Ornstein-Uhlenbeck dynamics. Namely, the law $P_{\beta,n}$ is invariant for the operator $$Lf(x)=\Delta f(x)-\nabla H(x)\cdot\nabla f(x)$$ where $$H(x)=n\frac{\beta}{4}(x_1^2+\cdots+x_n^2)-\frac{\beta}{2}\sum_{i\neq j}\log|x_i-x_j|.$$ Since $\partial_{x_i}H(x)=n\frac{\beta}{2}x_i-\beta\sum_{j\neq i}\frac{1}{x_i-x_j}$, we find $$L=\sum_{i=1}^n\partial_{x_i}^2-n\frac{\beta}{2}\sum_{i=1}^nx_i\partial_{x_i}+\frac{\beta}{2}\sum_{i\neq j}\frac{\partial_{x_i}-\partial_{x_j}}{x_i-x_j}.$$ The first two terms form an Ornstein-Uhlenbeck operator, while the last term leaves globally invariant symmetric polynomials. Certain symmetric polynomials are eigenvectors. For instance the function $x_1+\cdots+x_n$ is an eigenvector, indeed we find $$L(x_1+\cdots+x_n)=-n\frac{\beta}{2}(x_1+\cdots+x_n).$$. Similarly, the function $x_1^2+\cdots+x_n^2+c$ is, for a choice of $c$, an eigenvector since $$L(x_1^2+\cdots+x_n^2)=2n+\beta n(n-1)-n\beta(x_1^2+\cdots+x_n^2).$$
Let $S={(S_t)}_{t\geq0}$ be the stochastic process on $\mathbb{R}^n$ solution of the stochastic differential equation $$\mathrm{d}S_t=\sqrt{2}\mathrm{d}B_t-\nabla H(S_t)\mathrm{d}t$$ where $B={(B_t)}_{t\geq0}$ is a standard Brownian motion. The Itô formula gives, for $f\in\mathcal{C}^2(\mathbb{R}^n,\mathbb{R})$, $$\mathrm{d}f(S_t)=\sqrt{2}\nabla f(S_t)\cdot \mathrm{d}B_t+(Lf)(S_t)\mathrm{d}t.$$
With $f(x_1,\ldots,x_n)=x_1+\cdots+x_n$ we get that $U_t:=S_{t,1}+\cdots+S_{t,n}$ solves $$\mathrm{d}U_t=\sqrt{2 n}\mathrm{d}W_t-n\frac{\beta}{2}U_t\mathrm{d}t$$ where $W={(\frac{B_{t,1}+\cdots+B_{t,n}}{\sqrt{n}})}_{t\geq0}$ is a standard Brownian motion. Thus $U$ is an Ornstein-Uhlenbeck process. Since $U_\infty$ has the law of $X_n$, we recover by this way the formula $X_n\sim\mathcal{N}(0,2\beta^{-1})$.
With $f(x_1,\ldots,x_n)=x_1^2+\cdots+x_n^2$, we get that $V_t:=S_{t,1}^2+\cdots+S_{t,n}^2=|S_t|^2$ solves $$\mathrm{d}V_t=\sqrt{2}2\sqrt{V_t}\mathrm{d}W_t+n\beta\left(\frac{2}{\beta}+n-1-V_t\right)\mathrm{d}t$$ where $W={(\frac{S_t}{|S_t|}B_t)}_{t\geq0}$ is a standard Brownian motion. Thus $V$ is a Cox-Ingersoll-Ross process. The generator of such a process is a Laguerre operator, and the invariant distribution is a Gamma law. Since $V_\infty$ has the law of $|X_n|^2$, we recover the formula $|X_n|^2\sim\mathrm{Gamma}(…)$.
Complex case. For all $\beta>0$ and $n\geq2$, we consider the probability measure on $\mathbb{C}^n$ defined by
$$
\mathrm{d}P_{\beta,n}(x)
=\frac{\mathrm{e}^{-n\frac{\beta}{2}(|x_1|^2+\cdots+|x_n|^2)}}{C_{\beta,n}}\prod_{i<j}|x_i-x_j|^\beta\mathrm{d}x.
$$ Let $X_n=(X_{n,1},\ldots,X_{n,n})\sim P_{\beta,n}$. Up to our knowledge, there is no useful matrix model with independent entries valid for all $\beta$. However, it is possible as in the real case to use the eigenvectors of an (overdamped) Langevin dynamics, namely
$$
Lf(x)=\Delta f(x)-\nabla f(x)\cdot \nabla H(x)
$$ where $$
H(x)=n\frac{\beta}{2}(|x_1|^2+\cdots+|x_n|^2)-\frac{\beta}{4}\sum_{i\neq j}\log|x_i-x_j|^2.
$$
Now $\nabla_{x_i}H(x)=n\beta x_i-\beta\sum_{j\neq i}\frac{x_i-x_j}{|x_i-x_j|^2}$, which gives $$L=\sum_{i=1}^n\partial^2_{x_i}-n\beta\sum_{i=1}^nx_i\cdot\partial_{x_i}+\frac{\beta}{2}\sum_{j\neq i}\frac{(x_i-x_j)\cdot(\partial_{x_i}-\partial_{x_j})}{|x_i-x_j|^2}.$$ As in the real case, the first two terms still form an Ornstein-Uhlenbeck operator. Certain special symmetric polynomials are eigenvectors, such as $\Re(x_1+\cdots+x_n)$, $\Im(x_1+\cdots+x_n)$ and $|x_1|^2+\cdots+|x_n|^2+c$ for a suitable constant $c$. More precisely, we have $$ L(\Re(x_1+\cdots+x_n))=-n\beta\Re(x_1+\cdots+x_n)$$ and $$L(\Im(x_1+\cdots+x_n))=-n\beta\Im(x_1+\cdots+x_n).$$ Similarly we find
$$
L(|x_1|^2+\cdots+|x_n|^2)=4n-2n\beta(|x_1|^2+\cdots+|x_n|^2)+\beta n(n-1).
$$ Let $S={(S_t)}_{t\geq0}$ be the stochastic process on $\mathbb{R}^{2n}$ solution of the stochastic differential equation $$\mathrm{d}S_t=\sqrt{2}\mathrm{d}B_t-\nabla H(S_t)\mathrm{d}t$$ where $B={(B_t)}_{t\geq0}$ is a standard Brownian motion. By Itô formula, for all $f\in\mathcal{C}^2(\mathbb{R}^{2n},\mathbb{R})$, $$\mathrm{d}f(S_t)=\sqrt{2}\nabla f(S_t)\cdot \mathrm{d}B_t+(Lf)(S_t)\mathrm{d}t.$$
If $f(x_1,\ldots,x_n)=\Re(x_1+\cdots+x_n)$ then $U_t:=\Re(S_{t,1}+\cdots+S_{t,n})$ solves $$\mathrm{d}U_t=\sqrt{2 n}\mathrm{d}W_t-n\beta U_t\mathrm{d}t$$ where $W={(\frac{\Re(B_{t,1}+\cdots+B_{t,n})}{\sqrt{n}})}_{t\geq0}$ is a standard Brownian motion. Thus $U$ is an Ornstein-Uhlenbeck process. Since $U_\infty$ has the law of $\Re(X_{n,1}+\cdots+X_{n,n})$, we get that $$\Re(X_{n,1}+\cdots+X_{n,n})\sim\mathcal{N}(0,\beta^{-1}).$$ By doing the same for $\Im$, we get that $\Re(X_{n,1}+\cdots+X_{n,n})$ and $\Im(X_{n,1}+\cdots+X_{n,n})$ are independent and of same law $\mathcal{N}(0,\beta^{-1})$ and therefore $$X_{n,1}+\cdots+X_{n,n}\sim\mathcal{N}(0,\beta^{-1}I_2).$$
If $f(x_1,\ldots,x_n)=|x_1|^2+\cdots+|x_n|^2$ then $V_t:=|S_{t,1}|^2+\cdots+|S_{t,n}|^2=|S_t|^2$ solves $$\mathrm{d}V_t=\sqrt{2}2\sqrt{V_t}\mathrm{d}W_t+2n\beta\left(\frac{2}{\beta}+\frac{n-1}{2}-V_t\right)\mathrm{d}t$$ where $W={(\frac{S_t}{|S_t|}B_t)}_{t\geq0}$ is a standard Brownian motion. Thus $V$ is a Cox-Ingersoll-Ross process. The generator of such a process is a Laguerre operator, and the invariant distribution is a Gamma law. Since $V_\infty$ has the law of $|X_n|^2$, we obtain the formula $$|X_n|^2\sim\mathrm{Gamma}\left(n+\frac{\beta n(n-1)}{4},\frac{n\beta}{2}\right).$$ When $\beta=2$, this is compatible with the observation of Kostlan that $n|X_n|^2$ has the law of a sum of $n$ independent random variables of law $\mathrm{Gamma}(1,1),\ldots,\mathrm{Gamma}(n,1)$.
We can compute quickly the mean using the invariance of $P_{\beta,n}$ with respect to $L$, namely
$$
0=4n+\beta n(n-1)-2n\beta\mathbb{E}(|X_{n,1}|^2+\cdots+|X_{n,n}|^2)
$$
gives
$$
\mathbb{E}(|X_n|^2)=\mathbb{E}(|X_{n,1}|^2+\cdots+|X_{n,n}|^2)=\frac{2}{\beta}+\frac{n-1}{2}.
$$
Note. A squared Ornstein-Uhlenbeck (OU) process is a Cox-Ingersoll-Ross (CIR) process. In this sense CIR processes play for OU processes the role played for BM by squared Bessel processes.
Further reading.
- Chafaï & Lehec, On Poincaré and logarithmic Sobolev inequalities for a class of singular Gibbs measures, arXiv:1805.00708
- Bolley & Chafaï & Fontbona, Dynamics of a planar Coulomb gas, arXiv:1706.08776