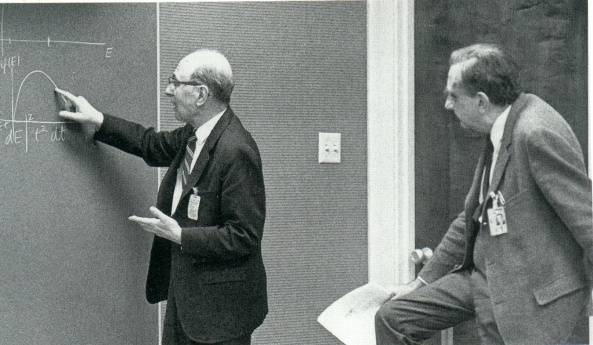
This tiny post is adapted from the introduction of a recent work with David García-Zelada and Paul Jung on the macroscopics and edge of a planar jellium seen as a Coulomb gas.
Potential theory. The Coulomb kernel $g$ in $\mathbb{R}^d$, $d\geq1$, is given for all $x\in\mathbb{R}^d$ by
\[ g(x)=\begin{cases}\displaystyle\log\frac{1}{|x|}&\text{if $d=2$}\\[1em]\displaystyle\frac{1}{(d-2)|x|^{d-2}}&\text{if $d\neq2$}\end{cases}.\] The Coulomb potential at point $x$ generated by a distribution of charges, say electrons, modeled by a probability measure $\mu$ on $\mathbb{R}^d$ is defined by \[U_\mu(x)=(g*\mu)(x)=\int g(x-y)\mathrm{d}\mu(y)\in(-\infty,+\infty].\] We have $U_\mu\in\mathrm{L}^1_{\mathrm{loc}}(\mathrm{d}x)$ and the identity $-\Delta g=c_d\delta_0$, where $c_d=d\omega_d$ is the surface of the unit ball and $\omega_d$ its volume, gives the inversion formula
\[-\Delta U_\mu=c_d\mu.\]In particular $U_\mu$ is super-harmonic in the sense that $\Delta U_\mu\leq0$ since $g$ is super-harmonic. The Coulomb self-interaction energy of the distribution of charges $\mu$ is defined when it makes sense by
\[\mathcal{E}(\mu)=\frac{1}{2}\iint g(x-y)\mathrm{d}\mu(x)\mathrm{d}\mu(y)=\frac{1}{2}\int U_\mu(x)\mathrm{d}\mu(x).\] Let $V:\mathbb{R}^d\to\mathbb{R}\cup\{+\infty\}$ be a lower semi-continuous function playing the role of an external potential, producing an external electric field $-\nabla V$. If $V$ grows faster than $g$ at infinity, the Coulomb energy $\mathcal{E}_V$ with external field is defined by \[\mu\mapsto\mathcal{E}_V(\mu)=\mathcal{E}(\mu)+\int V\mathrm{d}\mu.\]
Coulomb gases. A Coulomb gas in $\mathbb{R}^d$ with $n$ particles, potential $V$, and inverse temperature $\beta\geq0$ is the exchangeable Boltzmann–Gibbs law on $(\mathbb{R}^d)^n$ with density proportional to \[\mathrm{e}^{-\beta E_n(x_1,\ldots,x_n)}\quad\text{where}\quad E_n(x_1,\ldots,x_n)=\sum_{i<j}g(x_i-x_j)+n\sum_{i=1}^nV(x_i).\] It models a gas of unit charged particles, or more precisely a random configuration of unit charged particles. We should keep in mind that we play here with electrostatics rather than with electrodynamics and that thus we do not have a magnetic field.
Wigner jellium. Let us consider $n$ unit negatively charged particles (electrons) at positions $x_1,\ldots,x_n$ in $\mathbb{C}$, lying in a positive background of total charge $\alpha>0$ smeared according to a probability measure $\rho$ on $\mathbb{C}$ with finite Coulomb energy $c=\mathcal{E}(\rho)$. We could alternatively suppose that the particles are positively charged (ions) and the background is negatively charged (electrons), this reversed choice would not affect the analysis of the model. The total energy of the system, counting each pair a single time, is given by
\[\sum_{i<j}g(x_i-x_j)-\alpha\sum_{i=1}^nU_\rho(x_i)+\alpha^2c.\]
This matches the Coulomb energy of a Coulomb gas with $V=-\frac{\alpha}{n}U_\rho$. This observation leads us to define the jellium model on $S\subset\mathbb{R}^d$ with background charge $\alpha>0$ and background distribution $\rho$ with $\mathrm{supp}\rho\subset S$ as being the Coulomb gas on $\mathbb{R}^d$, with potential $V$ given by
\[V=\begin{cases}-\frac{\alpha}{n}U_\rho&\text{on $S$}\\+\infty&\text{on $S^c$}.\end{cases}\]
We say that the system is charge neutral. when $\alpha=n$. We say that it is uniform when $\rho$ is the uniform distribution on some compact subset of $\mathbb{R}^d$. The great majority of jellium models studied in the literature are charge neutral and satisfy $S=\mathrm{supp}\rho$.
Conversely, a Coulomb gas with sub-harmonic potential $V$ (meaning $\Delta V\geq0$) can be seen as a jellium with background \[\rho=\frac{\Delta V}{c_d\alpha}\mathrm{d}x\] on $S=\mathbb{R}^d$. When $V$ is not sub-harmonic then $\rho$ is no longer a positive measure but we can still interpret it as a background with opposite charge on $\{\Delta V<0\}$.
The complex Ginibre ensemble is a famous Coulomb gas with \[d=2\quad\text{and}\quad V=\left|\cdot\right|^2,\] for which $\Delta V$ is constant, leading to an interpretation of this Coulomb gas as a degenerate jellium on the full space with Lebesgue background. This Coulomb gas or Wigner jellium describes the eigenvalues of a Gaussian random complex $n\times n$ matrix $A$ with density proportional to $\exp(-\mathrm{Trace}(A\bar{A}^\top))$. Equivalently, the entries of $A$ are independent and identically distributed with independent real and imaginary parts having a Gaussian law of mean $0$ and variance $1/(2n)$. This gas or jellium also appears in various other places in the mathematical physics literature, for instance as the modulus of the wave function in Laughlin’s model of the fractional quantum Hall effect, in the description of the vortices in the Ginzburg-Landau model of superconductivity, and in a model of rotating trapped fermions.
The Forrester-Krishnapur spherical ensemble is another remarkable Coulomb gas with \[d=2\quad\text{and}\quad V=\Bigr(1+\frac{1}{n}\Bigr)\log(1+\left|\cdot\right|^2),\] for which $\Delta V=4(1+1/n)/(1+\left|\cdot\right|^2)^2$, leading to an interpretation of this Coulomb gas as a jellium on the full space with a heavy tailed background. The name of this gas comes from the fact that it is the image by the stereographical projection of the Coulomb gas on the sphere, with constant potential, onto to the complex plane. This Coulomb gas describes the eigenvalues of $AB^{-1}$ where $A$ and $B$ are two independent copies of complex Ginibre random matrices. We can loosely interpret $AB^{-1}$ as a sort of matrix analogue of the Cauchy distribution since when $A$ and $B$ are $1\times 1$ matrices, this is precisely a Cauchy distribution.
We can also consider such a background-potential inverse problem for the one-dimensional log-gases of random matrix theory, which can be seen as two-dimensional Coulomb gases confined to the real line, such as the Gaussian Unitary Ensemble. For instance it follows from the discussion in Peter Forrester book that the logarithmic potential of the density
\[
x\mapsto\frac{\sqrt{2n}}{\pi}\sqrt{1-\frac{x^2}{2n}}\mathbf{1}_{|x|<\sqrt{2n}}
\]
is given on the interval $S=[-\sqrt{2n},\sqrt{2n}]$ by
\[
x\mapsto \frac{x^2}{2}+\frac{n}{2}\Bigr(\log\frac{n}{2}-1\Bigr).
\]
A bit of history. The jellium model was used around 1938 by Eugene P. Wigner in a famous article for the modeling of electrons in metals, more than ten years before his renowned works on random matrices. This model was inspired from the Hartree-Fock model of quantum mechanics, see GV, LN, LS, S1, and LLS1, LLS2. The term jellium was apparently coined by Conyers Herring since the smeared charge could be viewed as a positive jelly, see H1. The model is also known as a one-component plasma with background. As already mentioned, usually charge-neutral jellium models are studied, and this is done typically after restricting the electrons to live on some compact support of positive background. The restriction ensures integrability of the energy and the interest is usually focused on the distribution/behavior of electrons in the bulk of the limiting system when the volume of the compact set goes to infinity (thermodynamic limit). There are some exceptions where the edge has been considered, for instance in CFTW. Also, the edge of Laughlin states has been considered in CFTW and GJA.
The case $d=3$ is considered by Lieb and Narnhofer, and quoting them: “It is also possible to consider the one- and two-dimensional versions of this problem, where the Coulomb potential $|x|^{-1}$ is replaced by $-|x|$ and $-\log|x|$, respectively. In the one-dimensional, classical case, Baxter calculated the partition function exactly. For that case, Kunz showed that the one-particle distribution function exists and that it has crystalline ordering, i.e., the Wigner lattice exists for all temperatures. Brascamp and Lieb showed the same to be true in the quantum mechanical case for one-component fermions when $\beta$ is large enough. Although we do not deal with the one-dimensional problem here, our methods would apply in that case. In two dimensions there are difficulties connected with the long-range nature of the $-\log|x|$ potential, and we shall not discuss this here.” For more background literature on the jellium, see also AJ, JLM, F, AJJ, JJ, CDFLV. See in particular LWL for the fluctuations of non-neutral jelliums.
Coulomb gas models appeared naturally in statistics around 1920-1930 in the study of the spectrum of empirical covariance matrices of Gaussian samples. Nowadays we speak about the Laguerre ensemble and Wishart random matrices. This was almost ten years before the introduction of the jellium model by Wigner. In the 1950’s, Wigner rediscovered, by accident, these works by reading a statistics textbook, and this motivated him to use random matrices for the modeling of energy levels of heavy nuclei in atomic physics, see this former blog-post. We refer to Bohigas and Weidenmüller for these historical aspects. The work of Wigner was amazingly successful, and he received in 1963 a Nobel prize in Physics “for his contributions to the theory of the atomic nucleus and the elementary particles, particularly through the discovery and application of fundamental symmetry principles”. The term Coulomb gas is explicitly used by Dyson in his first seminal 1962 paper and by Ginibre in his 1965 paper. The term Fermi-gas is also used.