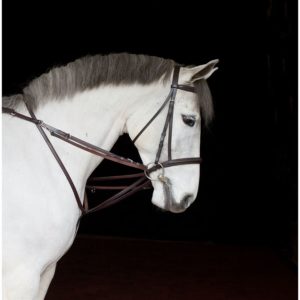
This post is inspired from the exam of my master course on stochastic calculus. The processes considered in this post are in continuous time, defined on a filtered probability space \( {(\Omega,\mathcal{F},{(\mathcal{F}_t)}_{t\geq0},\mathbb{P})} \), adapted, and have almost surely continuous trajectories.
Local martingales. If \( {{(M_t)}_{t\geq0}} \) is a martingale, the Doob stopping theorem states that if \( {T} \) is a stopping time, then the stopped process \( {{(M_{t\wedge T})}_{t\geq0}} \) is again a martingale.
Stopping can be used in general to truncate the trajectories of a process with a cutoff, in order to gain more integrability or tightness, while keeping adaptation and continuity. Typically if \( {{(X_t)}_{t\geq0}} \) is an adapted process, we could consider the sequence of stopping times \( {{(T_n)}_{n\geq0}} \) defined by
\[ T_n=\inf\{t\geq0:|X_t|\geq n\}, \]
which satisfies almost surely \( {T_n\nearrow+\infty} \) as \( {n\rightarrow\infty} \) and for which for all \( {n} \) the stopped process \( {{(X_{t\wedge T_n})}_{t\geq0}} \) is bounded by \( {|X_0|\vee n} \). Since \( {X} \) is continuous, almost surely, for all \( {t\geq0} \), \( {\lim_{n\rightarrow\infty}X_{t\wedge T_n}=X_t} \). We say that \( {{(T_n)}_{n\geq0}} \) is a localizing sequence.
Now a local martingale is simply an adapted processes \( {{(X_t)}_{t\geq0}} \) such that for all \( {n\geq0} \) the stopped process \( {{(X_{t\wedge T_n})}_{t\geq0}} \) is a (bounded) martingale.
Every martingale is a local martingale. However the converse is false, and strict local martingales do exist. We give below one of the most famous example. Local martingales also popup naturally in the construction of the Itô stochastic integral. We give below a simple example of a stochastic integral which is a strict local martingale.
Domination. If \( {{(X_t)}_{t\geq0}} \) is a local martingale which is dominated by an integrable random variable, in the sense that \( {\mathbb{E}\sup_{t\geq0}|X_t|<\infty} \), then \( {{(X_t)}_{t\geq0}} \) is a martingale, and in fact a uniformly integrable martingale. Namely, for all \( {t\geq0} \) and all \( {s\in[0,t]} \), by the martingale property for the stopped process and dominated convergence,
\[ \begin{array}{rcl} X_s &=&\lim_{n\rightarrow\infty}X_{s\wedge T_n}\\ &=&\lim_{n\rightarrow\infty}\mathbb{E}(X_{t\wedge T_n}\mid\mathcal{F}_s)\\ &=&\mathbb{E}(\lim_{n\rightarrow\infty}X_{t\wedge T_n}\mid\mathcal{F}_s)\\ &=&\mathbb{E}(X_t\mid\mathcal{F}_s). \end{array} \]
Therefore strict local martingales are not dominated, their supremum is not integrable. However strict local martingales can be uniformly integrable, and even bounded in \( {\mathrm{L}^2} \).
A strict local martingale bounded in \( {\mathrm{L}^2} \). Let \( {{(B_t)}_{t\geq0}} \) be a standard Brownian motion in \( {\mathbb{R}^3} \) issued from \( {x\in\mathbb{R}^3} \) with \( {x\neq0} \). Then the inverse Bessel process \( {{(|B_t|^{-1})}_{t\geq0}} \) is a well defined local martingale, bounded in \( {\mathrm{L}^2} \), but is not a martingale.
Proof. Our first goal is to show that the process \( {{(|B_t|^{-1})}_{t\geq0}} \) is well defined, namely that \( {B} \) takes its values in \( {D=\{x\in\mathbb{R}^3:x\neq0\}} \). For that we consider \( {0<r<|x|} \), and we define
\[ T_r=\inf\{t\geq0:|x+B_t|=r\}. \]
The stopped process \( {{(X_t)}_{t\geq0}={(B_{t\wedge T_r})}_{t\geq0}} \) takes its values in the open set \( {D} \). Now, the function \( {y\in D\mapsto u(y)=|y|^{-1}} \) is harmonic, namely \( {\Delta u=0} \) since
\[ \partial_iu(y)=-\frac{y_i}{|y|^3}, \quad\text{and}\quad \partial^2_{i,i}u(y)=\frac{3}{2}\frac{|y|^3-3y_i^2|y|}{|y|^{5}}. \]
Also, by the Itô formula, for all \( {t\geq0} \), using \( {\langle X^j,X^k\rangle_t=\langle B^j,B^k\rangle_{t\wedge T_r}=(t\wedge T_r)\mathbf{1}_{j=k}} \),
\[ u(X_t)=u(X_0)+\int_0^t\nabla u(X_s)\mathrm{d}X_s+\frac{1}{2}\int_0^{t\wedge T_r}\Delta u(X_s)\mathrm{d}s. \]
The last integral vanishes because \( {\Delta u=0} \) on \( {D} \), hence
\[ {(u(X_t))}_{t\geq0}={(|B_{t\wedge T_r}|^{-1})}_{t\geq0} \]
is a local martingale, bounded by the constant \( {r^{-1}} \), thus it is a bounded martingale.
Let us compute now \( {\mathbb{P}(T_r<T_R)} \) for \( {0<r<|x|<R} \). Since a \( {1} \)-dimensional Brownian motion almost surely escapes from every finite interval, the first component of our \( {3} \)-dimensional Brownian motion started from \( {x} \) almost surely escapes from \( {[-R,R]} \), and it follows that almost surely \( {T_R<\infty} \). In particular almost surely either \( {T_r<T_R} \) or \( {T_r>T_R} \) and we cannot have \( {T_r=T_R} \). Next, we have first the immediate equation
\[ 1=\mathbb{P}(T_r<T_R)+\mathbb{P}(T_r>T_R). \]
On the other hand, by the Doob stopping theorem for the bounded martingale \( {Y={(|B_{t\wedge T_r}|^{-1})}_{t\geq0}} \) and the finite stopping time \( {T_R} \), we get the new equation
\[ \frac{1}{|x|} =\mathbb{E}(Y_0) =\mathbb{E}(Y_{T_R}) =\mathbb{E}\left(\frac{1}{|B_{T_r\wedge T_R}|}\right) =\frac{\mathbb{P}(T_r<T_R)}{r}+\frac{\mathbb{P}(T_r>T_R)}{R}. \]
Solving this couple of equations gives
\[ \mathbb{P}(T_r<T_R)=\frac{R^{-1}-|x|^{-1}}{R^{-1}-r^{-1}}. \]
Now \( {T_r<T_R} \) if \( {R>\sup_{s\in[0,B_{T_r}]}|B_s|} \), hence \( {\{T_r<T_R\}\underset{R\rightarrow\infty}{\nearrow}\{T_r<\infty\}} \). It follows that
\[ \mathbb{P}(T_r<T_R) \underset{R\nearrow\infty}{\nearrow} \mathbb{P}(T_r<\infty) \]
and thus, from the formula above,
\[ \mathbb{P}(T_r<\infty) =\lim_{R\rightarrow\infty}\mathbb{P}(T_r<T_R) =\frac{|x|^{-1}}{r^{-1}}=\frac{r}{|x|}. \]
Now almost surely \( {B} \) is continuous and therefore \( {\{T_r<\infty\}\underset{r\searrow0^+}{\searrow}\{T_0<\infty\}} \) and thus
\[ \mathbb{P}(T_0<\infty) =\lim_{r\rightarrow0^+}\mathbb{P}(T_r<\infty) =\lim_{r\rightarrow0^+}\frac{r}{|x|}=0. \]
Therefore \( {B} \) takes its values in \( {D} \), and the process \( {{(|B_t|^{-1})}_{t\geq0}} \) is well defined. This process is also adapted. It is a local martingale, localized by \( {T_r} \) with \( {r\in\{(|x|+n)^{-1}:n\geq1\}} \).
Let us show that \( {\lim_{t\rightarrow\infty}|B_t|=+\infty} \) almost surely. This is typical to dimension \( {d\geq3} \), related to transcience of Brownian motion. Indeed, since \( {{(|B_t|^{-1})}_{t\geq0}} \) is a non-negative local martingale, it is a super-martingale. This is easily seen by using a localization sequence and the Fatou lemma. It follows that almost surely it converges as \( {t\rightarrow\infty} \) to an integrable random variable, hence, almost surely \( {\lim_{t\rightarrow\infty}|B_t|} \) exists in \( {[0,+\infty]} \), and the convergence holds also in law and the limiting law can only be \( {\delta_\infty} \).
Let us show now that \( {{(|B_t|^{-1})}_{t\geq0}} \) is bounded in \( {\mathrm{L}^2} \). By rotational invariance and scaling of Brownian motion, we can assume without loss of generality that \( {x=(1,0,0)} \). Since \( {B_t\sim\mathcal{N}(x,tI_3)} \), using spherical coordinates
\[ y_1=r\cos(\theta)\sin(\varphi),\quad y_2=r\sin(\theta)\sin(\varphi),\quad y_3=r\cos(\varphi) \]
with \( {r\in[0,\infty)} \), \( {\theta\in[0,2\pi)} \), \( {\varphi\in[0,\pi)} \), we have \( {\mathrm{d}y=r^2\sin(\varphi)\mathrm{d}r\mathrm{d}\theta\mathrm{d}\varphi} \), and for all \( {t>0} \),
\[ \begin{array}{rcl} \mathbb{E}(|B_t|^{-2}) &=&(2\pi t)^{-3/2}\int_{\mathbb{R}^3} \left|y\right|^{-2}\mathrm{e}^{-\frac{y_1^2+y_2^2+(y_3-1)^2}{2t}}\mathrm{d}y\\ &=&(2\pi t)^{-3/2}\int_0^\infty\int_0^{2\pi}\int_0^\pi r^{-2}\mathrm{e}^{-\frac{r^2\sin(\varphi)^2+(r\cos(\varphi)-1)^2}{2t}} r^2\sin(\varphi)\mathrm{d}r\mathrm{d}\theta\mathrm{d}\varphi\\ &=&(2\pi)^{-1/2}t^{-3/2}\int_0^\infty\int_0^\pi \mathrm{e}^{-\frac{r^2\sin(\varphi)^2+(r\cos(\varphi)-1)^2}{2t}} \sin(\varphi)\mathrm{d}r\mathrm{d}\varphi\\ &=&(2\pi)^{-1/2}t^{-3/2}\int_0^\infty\int_0^\pi \mathrm{e}^{-\frac{r^2-2r\cos(\varphi)+1}{2t}} \sin(\varphi)\mathrm{d}r\mathrm{d}\varphi\\ &=&(2\pi)^{-1/2}t^{-3/2}\mathrm{e}^{-\frac{1}{2t}}\int_0^\infty\mathrm{e}^{-\frac{r^2}{2t}}\Bigr(\int_{-1}^1 \mathrm{e}^{\frac{ru}{t}}\mathrm{d}u\Bigr)\mathrm{d}r\\ &=&(2\pi)^{-1/2}t^{-3/2}\mathrm{e}^{-\frac{1}{2t}}\int_0^\infty\mathrm{e}^{-\frac{r^2}{2t}}\Bigr[\frac{t}{r}\mathrm{e}^{\frac{ru}{t}}\Bigr]_{u=-1}^{u=1}\mathrm{d}r\\ &=&2(2\pi)^{-1/2}t^{-3/2}\mathrm{e}^{-\frac{1}{2t}}\int_0^\infty \mathrm{e}^{-\frac{r^2}{2t}}\frac{\sinh(\frac{r}{t})}{\frac{r}{t}}\mathrm{d}r\\ &=&2(2\pi)^{-1/2}t^{-3/2}\mathrm{e}^{-\frac{1}{2t}} \sum_{n=0}^\infty\frac{1}{(2n+1)!}\int_0^\infty\left(\frac{r}{t}\right)^{2n}\mathrm{e}^{-\frac{r^2}{2t}}\mathrm{d}r\\ &=&t^{-1}\mathrm{e}^{-\frac{1}{2t}}\sum_{n=0}^\infty\frac{t^{-2n}}{(2n+1)!}(2\pi t)^{-1/2}\int_{-\infty}^\infty r^{2n}\mathrm{e}^{-\frac{r^2}{2t}}\mathrm{d}r\\ &=&t^{-1}\mathrm{e}^{-\frac{1}{2t}}\sum_{n=0}^\infty\frac{t^{-2n}}{(2n+1)!}t^n\frac{(2n-1)!}{2^{n-1}(n-1)!}\\ &=&t^{-1}\mathrm{e}^{-\frac{1}{2t}}\sum_{n=0}^\infty\frac{(2t)^{-n}}{(2n+1)n!}\\ &=&2\mathrm{e}^{-\frac{1}{2t}} \sum_{n=0}^\infty\frac{(2t)^{-(n+1)}}{(2n+1)n!}\\ &\leq&2\mathrm{e}^{-\frac{1}{2t}} \sum_{n=0}^\infty\frac{(2t)^{-(n+1)}}{(n+1)!} =2\mathrm{e}^{-\frac{1}{2t}}(\mathrm{e}^{\frac{1}{2t}}-1)\leq2. \end{array} \]
Let us show that \( {{(Z_t)}_{t\geq0}={(|B_t|^{-1})}_{t\geq0}} \) is not a martingale by contradiction. Assume that it is a martingale. Since it is bounded in \( {\mathrm{L}^2} \), \( {\lim_{t\rightarrow\infty}Z_t=Z_\infty} \) almost surely and in \( {\mathrm{L}^1} \), with \( {Z_\infty\geq0} \) and \( {Z_\infty\in\mathrm{L}^1} \). Moreover \( {\mathbb{E}(Z_\infty)=\mathbb{E}(Z_0)=|x|^{-1}>0} \). But almost surely \( {\lim_{t\rightarrow\infty}|B_t|=+\infty} \), hence almost surely \( {Z_\infty=0} \), thus \( {\mathbb{E}(Z_\infty)=0} \), a contradiction.
Alternatively, we could use Doob stopping for u.i. martingales with the u.i. martingale \( {Z} \) and the finite stopping time \( {T_R} \), which gives \( {|x|^{-1}=\mathbb{E}(Z_0)=\mathbb{E}(Z_{T_R})=R^{-1}} \), a contradiction.
Alternatively, we could conduct explicit computations to show that \( {\mathbb{E}(Z_t)\searrow0} \) as \( {t\rightarrow\infty} \), which is thus yet another way to show that \( {{(Z_t)}_{t\geq0}} \) is not a martingale!
Stochastic differential equation. Actually \( {{(Z_t)}_{t\geq0}={(|B_t|^{-1})}_{t\geq}} \) solves
\[ Z_t=\frac{1}{|x|}-\int_0^tZ_s^2\mathrm{d}W_s. \]
Itô stochastic integrals. Let us give an example of an Itô stochastic integral which is a local martingale but not a martingale. Of course we could consider the trivial example \( {\int_0^t\mathrm{d}Z_s=Z_t-Z_0} \) where \( {{(Z_t)}_{t\geq0}={(|B_t|^{-1})}_{t\geq0}} \) is the strict local martingale considered previously, but a deeper understanding is expected here!
A more interesting idea relies on the stochastic integral
\[ I_B(\varphi)=\int_0^\bullet\varphi_s\mathrm{d}B_s \]
where \( {\varphi} \) is the single step function \( {\varphi=U\mathbf{1}_{(0,1]}} \) with \( {U} \) \( {\mathcal{F}_0} \)-measurable. A property of the Itô stochastic integral for semi-martingale integrators (here \( {B} \)) gives
\[ I_B(\varphi) =UB_{\bullet\wedge 1}-UB_0 =UB_{\bullet\wedge 1}. \]
Now if we take \( {U} \) independent of \( {B} \), then, in \( {[0,+\infty]} \),
\[ \mathbb{E}(|I_B(\varphi)_1|) =\mathbb{E}(|U|)\mathbb{E}(|B_1|). \]
Thus, if \( {U} \) is not integrable then \( {I_B(\varphi)_1} \) is not integrable, and \( {I_B(\varphi)} \) is not a martingale.
4 Comments