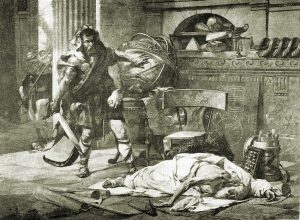
This post is about the semicircle distribution, and the way it appears in special unitary $2\times 2$ matrices. The link passes through the sphere $S^3$ and the Archimedes theorem.
Semicircle. The semicircle distribution on the interval $[-2\sigma,2\sigma]$, $\sigma > 0$, is \[ \mathrm{d}\mu^{\mathrm{SC}}_\sigma(x) =\frac{\sqrt{4\sigma^2-x^2}}{2\pi\sigma^2}\mathbf{1}_{x\in[-2\sigma,2\sigma]}(x)\mathrm{d}x. \] It has mean $0$ and variance $\sigma^2$. It is a symmetric $\mathrm{Beta}_{[-2\sigma,2\sigma]}(\frac{3}{2},\frac{3}{2})$ distribution since \[ \sqrt{4\sigma^2-x^2}=(2\sigma-x)^{\frac{3}{2}-1}(2\sigma+x)^{\frac{3}{2}-1}. \] It is the projection on $\mathbb{R}$ of the uniform distribution on the disc of radius $2\sigma$.
Archimedes and projection of $S^3$. The famous extended Archimedes theorem on the sphere and the cylinder states that if $(U_1,\ldots,U_{n+2})$ is uniformly distributed on the unit sphere $S^{n+1}=\{x\in\mathbb{R}^{n+2}:x_1^2+\cdots+x_{n+2}^2=1\}$ of $\mathbb{R}^{n+2}$, then its projection $(U_1,\ldots,U_n)$ on $\mathbb{R}^n$ is uniformly distributed on the unit ball $\{x\in\mathbb{R}^n:x_1^2+\cdots+x_n^2\leq1\}$. In particular, the projection on $\mathbb{R}^2$ of the uniform distribution on $S^3$ is the uniform distribution on the unit disc. Also its projection on $\mathbb{R}$ is the semicircle distribution $\mu^{\mathrm{SC}}_{\frac{1}{2}}$.
Let us retain that if a random vector is uniformly distributed on $S^3$ then each of its $4$ coordinates are distributed according to the semicircle distribution $\mu^{\mathrm{SC}}_{\frac{1}{2}}$.
Note that $S^3$ can also be understood as the quaternionic unit sphere via \[ |x|=\sqrt{x_1^2+x_2^2+x_3^2+x_4^2} \quad\text{where}\quad x=x_1+x_2\mathrm{i}+x_3\mathrm{j}+x_4\mathrm{k}\in\mathbb{H}\equiv\mathbb{R}^4, \] just like $S^1$ and $S^2$ are the unit spheres of $\mathbb{R}$ and $\mathbb{C}$ respectively.
$S^3$ is diffeomorphic to $\mathrm{SU}(2)$. The special unitary group $\mathrm{SU}(2)$ is the set of $2\times 2$ unitary matrices with determinant equal to $1$. Let us write $U\in\mathrm{SU}(2)$ as \[ U=\begin{pmatrix} a & c\\ b & d\end{pmatrix},\quad a,b,c,d\in\mathbb{C}. \] The condition $U\in\mathrm{U}(2)$ gives $|a|^2+|b|^2=1$, $|c|^2+|d|^2=1$, and $(c,d)\perp(a,b)$, hence $(c,d)=\mathrm{e}^{\mathrm{i}\theta}(-\overline{b},\overline{a})$, for some phase $\theta\in[0,2\pi)$. Next, the unit determinant condition reads $1=ad-bc=(|a|^2+|b|^2)\mathrm{e}^{\mathrm{i}\theta}$, hence $\theta=0$. We have obtained the parametrization \[ U=\begin{pmatrix} a & -\overline{b}\\ b & \overline{a}\end{pmatrix},\quad a,b\in\mathbb{C},\quad |a|^2+|b|^2=1. \] In other words $\mathrm{SU}(2)$ is diffeomorphic to $S^3$, which is also the quaternionic unit sphere. The uniform probability measure on $S^3$ gives the uniform probability measure on $\mathrm{SU}(2)$, which is the normalized Haar measure on this group. Also, combining with what we have already seen on $S^3$, if $U$ is a random matrix distributed according to the normalized Haar measure on $\mathrm{SU}(2)$, then $\Re U_{11}$ follows the semicircle distribution $\mu^{\mathrm{SC}}_{\frac{1}{2}}$.
Eigenvalues of $\mathrm{SU}(2)$. If $U\in\mathrm{SU}(2)$, then its eigenvalues $\lambda_1$ and $\lambda_2$ belong to the unit circle of the complex plane, while $1=\det(U)=\lambda_1\lambda_2$, hence $\lambda_1=\mathrm{e}^{\mathrm{i}\theta}$ and $\lambda_2=\mathrm{e}^{-\mathrm{i}\theta}$, for some $\theta\in[0,\pi)$. Thus, using the fact that $U_{22}=\overline{U_{11}}$, we get \[ 2\Re U_{11}=\mathrm{Tr}(U)=2\cos(\theta). \] It follows that if $U$ is a random matrix distributed according to the normalized Haar measure on $\mathrm{SU}(2)$, then $\mathrm{Tr}(U)=2\cos(\theta)$ follows the semicircle distribution $\mu^{\mathrm{SC}}_1$.
Conjugacy classes of $\mathrm{SU}(2)$. Two elements $U,V\in\mathrm{SU}(2)$ have same conjugacy class iff $U=WVW^*$ for some $W\in\mathrm{SU}(2)$. This is equivalent to say that they have the same eigenvalues $\{\mathrm{e}^{\pm\mathrm{i}\theta}\}$, in other words, the same trace, as seen previously. If we parametrize the set of conjugacy classes by the trace, then it follows the semicircle distribution $\mu^{\mathrm{SC}}_1$.
Elliptic curves and Sato-Tate conjecture. Formulated independently by Mikio Sato (1928 - 2023) and John Torrence Tate Jr (1925 - 2019) around 1960, it states that for any fixed elliptic curve over the rationals, without complex multiplication, denoting $N$ its conductor or complexity, $N_p$ the number of its points mod $p$, $\mathcal{P}_n$ the set of prime numbers $\leq n$ not dividing $N$, and $|\mathcal{P}_n|$ its cardinal, we have the asymptotic equipartition \[ \frac{1}{|\mathcal{P}_n|}\sum_{p\in\mathcal{P}_n}\delta_{a_p} \xrightarrow[n\to\infty]{\mathrm{weak}} \mu^{\mathrm{SC}}_1 \quad\text{where}\quad a_p=\frac{(p+1)-N_p}{\sqrt{p}}. \] The weak (or narrow) convergence is with respect to continuous and bounded test functions, namely the convergence in law of probability theory. The link with $\mathrm{SU}(2)$ is via the interpretation of $a_p$ as a normalized trace of a Frobenius endomorphism. This conjecture was essentially proved around 2008 by Laurent Clozel (1953 - ), Michael Harris (1954 - ), Nicholas Shepherd-Barron (1955 - ), and Richard Taylor (1962 - ).
Elliptic curves are fascinating objects from algebraic number theory, connected to modular form by the Shimura-Taniyama correspondence, after Gorō Shimura (1930 - 2019) and Yutaka Taniyama (1927 - 1958), at the heart of the proof by Andrew Wiles (1953 - ) and Richard Taylor (1962 - ) of the last theorem of Pierre de Fermat (196? - 1665). They are also used in cryptography - in particular for cryptocurrencies such as Bitcoin - an application that motivated a conjecture formulated in 1976 by Serge Lang (1927 - 2005) and Hale Trotter (1931 - 2022).
Further reading.
- On this blog
Archimedes theorem on sphere and cylinder
March 18, 2024 - Henri Carayol
La conjecture de Sato-Tate, d'après Clozel, Harris, Shepherd-Barron, Taylor
Séminaire Bourbaki, Exposé n°977 (2007) - Michael Harris
The Sato-Tate conjecture
Introductory course for doctoral students, available online (2007) - Andrew V. Sutherland
Sato-Tate Distributions
Contemporary Mathematics 740 197-248 (2019) - Lawrence C. Washington
Elliptic curves: number theory and cryptography
Chapman and Hall/CRC (2008)
Final comment. Henri Carayol (1953 - ) is a French number‑theorist at Strasbourg. He should not be confused with Michel Charles Henri Carayol (1934 - 2003), the CEA engineer‑physicist who is one of the fathers of the French hydrogen bomb.
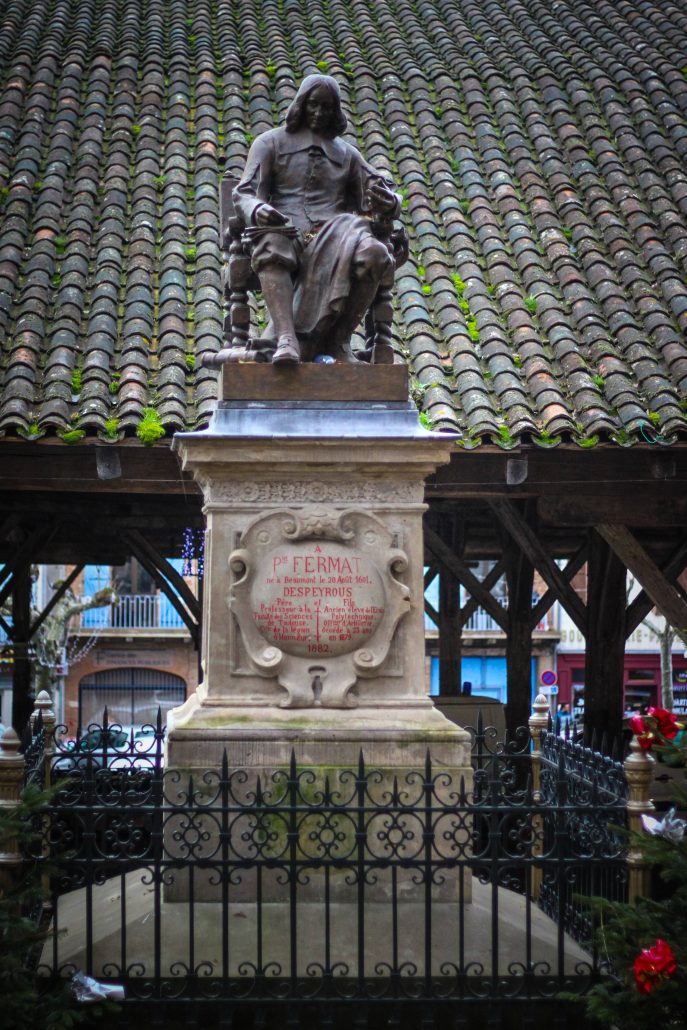