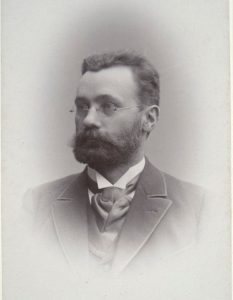
Even if the Mellin transform is just a deformed Fourier or Laplace transform, it plays a pleasant role in all the mathematics involving powers and integral transforms, such as Dirichlet series in number theory, Riesz power kernels in potential theory, mathematical statistics, etc. In particular, the famous Meijer G-function is defined as the inverse Mellin transform of ratios of products of Gamma functions. This tiny post is about an application of the Mellin transform to Riesz integral formulas, taken from an article by Bartłomiej Dyda, Alexey Kuznetsov, and Mateusz Kwaśnicki.
The Mellin transform and its inverse. Quoting Davies's book on integral transforms (chapter 12), we recall that the Fourier transform pair may be written in the form\begin{align}
A(\theta)&:=\int_{\mathbb{R}}a(t)\mathrm{e}^{\mathrm{i}\theta t}\mathrm{d}t,\quad\alpha<\Im\theta<\beta,\\
a(t)&=\frac{1}{2\pi}\int_{\mathrm{i}c+\mathbb{R}}A(\theta)\mathrm{e}^{-\mathrm{i}\theta t}\mathrm{d}\theta,\quad\alpha<c<\beta.
\end{align} The Mellin transform and its inverse follows if we introduce the variable change
\begin{equation}
z=\mathrm{i}\theta,\quad x=\mathrm{e}^t,\quad f(x)=a(\log(x)),
\end{equation} so that we obtain the reciprocal pair of integral transforms, for $f:(0,+\infty)\to\mathbb{R}$,
\begin{align}
F(z)&:=\int_0^\infty f(x)x^{z-1}\mathrm{d}x,\quad\alpha<\Re z<\beta,\\
f(x)&=\frac{1}{2\pi\mathrm{i}}\int_{c+\mathrm{i}\mathbb{R}}F(z)x^{-z}\mathrm{d}z,\quad\alpha<c<\beta.
\end{align} These are the Mellin transform, and the Mellin inversion formula. The integral defining the transform normally exists only in the strip $\alpha<\Re(z)<\beta$; therefore the inversion contour must be placed in this strip. For convenience we also denote by $\mathcal{M}f=F$ the Mellin transform of $f$.
The Mellin transform of $x\mapsto\mathrm{e}^{-x}$ is the Euler $\Gamma$ function. Its poles are $0,-1,-2,-3,\ldots$ In the same spirit, the Mellin transform of $x\mapsto(1-x)_+^{b-1}$ at point $z$ is $$\int_0^1x^{z-1}(1-x)^{b-1}\mathrm{d}x=\mathrm{Beta}(z,b).$$Recall the definition of the Euler Beta function $\mathrm{Beta}(a,b):=\int_0^1t^{a-1}(1-t)^{b-1}\mathrm{d}t=\frac{\Gamma(a)\Gamma(b)}{\Gamma(a+b)}$.
Lemma (Riesz potential of radial functions). Suppose that
\[
x\in\mathbb{R}^d\mapsto f(x)=\varphi(|x|^2)
\] where $\varphi:\mathbb{C}\to\mathbb{R}$ is given as the absolutely convergent inverse Mellin transform
\[
\varphi(r):=\frac{1}{2\pi\mathrm{i}}
\int_{\lambda+\mathrm{i}\mathbb{R}}
\mathcal{M}\varphi(z)r^{-z}\mathrm{d}z,
\quad
\text{for some }\lambda\in\mathbb{R}.
\] If $0<\alpha<2\lambda<d$ then the Riesz potential $(\left|\cdot\right|^{-(d-\alpha)}*f)(x)$ is well defined for $x\neq0$, and
\[
(\left|\cdot\right|^{-(d-\alpha)}*f)(x)
=\psi(|x|^2)
\] where \[
\psi(r):=
\frac{1}{2\pi\mathrm{i}}
\frac{\pi^{\frac{d}{2}}\Gamma(\frac{\alpha}{2})}{\Gamma(\frac{d-\alpha}{2})}
\int_{\lambda-\frac{\alpha}{2}+\mathrm{i}\mathbb{R}}
\frac{\Gamma(z)\Gamma(\frac{d-\alpha}{2}-z)}
{\Gamma(\frac{\alpha}{2}+z)\Gamma(\frac{d}{2}-z)}
\mathcal{M}\varphi(z+\tfrac{\alpha}{2})
r^{-z}\mathrm{d}z.
\] In other words, the Mellin transform of $\psi$ satisfies
\[
\mathcal{M}\psi(z)
=
\frac{\pi^{\frac{d}{2}}\Gamma(\frac{\alpha}{2})}
{\Gamma(\frac{d-\alpha}{2})}
\frac{\Gamma(z)\Gamma(\frac{d-\alpha}{2}-z)}
{\Gamma(\frac{\alpha}{2}+z)\Gamma(\frac{d}{2}-z)}
\mathcal{M}\varphi(z+\tfrac{\alpha}{2}).
\]
Proof of Lemma (Riesz potential of radial functions). The Lemma is Proposition 2 in Dyda-Kuznetsov-Kwaśnicki with $V\equiv1$ and $l=0$. The idea is to use the inverse Mellin transform of $\varphi$ to reduce the problem, via the Fubini theorem, to the Riesz potential of inverse powers of the norm, which is immediate from the semigroup property of the Riesz kernel. Namely, following eq. (1.1.12) in Landkof's book or eq. (8) p. 118 in Stein's book, on $\mathbb{R}^d$, the semigroup property for Riesz kernels reads, for all $\alpha,\beta\in\mathbb{C}$ such that $\Re\alpha,\Re\beta>0$ and $\Re\alpha+\Re\beta<d$, \begin{equation}\left|\cdot\right|^{-(d-\alpha)}*\left|\cdot\right|^{-(d-\beta)}
=\frac{c_d(\alpha)c_d(\beta)}{c_d(\alpha+\beta)}
\left|\cdot\right|^{-(d-(\alpha+\beta))}
\quad\text{where}\quad
c_d(z)
:=\frac{2^z\pi^{\frac{d}{2}}\Gamma(\frac{z}{2})}{\Gamma(\frac{d-z}{2})}.
\end{equation}Now, by the inverse Mellin transform of $\varphi$, the Fubini theorem, and the semigroup property,
\begin{align*}
(\left|\cdot\right|^{-(d-\alpha)}*f)(x)
&=\frac{1}{2\pi\mathrm{i}}
\int_{\lambda+\mathrm{i}\mathbb{R}}
(\left|\cdot\right|^{-(d-\alpha)}*\left|\cdot\right|^{-2z})\mathcal{M}\varphi(z)\mathrm{d}z\\
&=\frac{1}{2\pi\mathrm{i}}
\int_{\lambda+\mathrm{i}\mathbb{R}}
\frac{c_d(\alpha)c_d(d-2z)}{c_d(d+\alpha-2z)}
\mathcal{M}\varphi(z)\left|\cdot\right|^{-(2z-\alpha)}\mathrm{d}z\\
&=\frac{1}{2\pi\mathrm{i}}
\int_{\lambda-\frac{\alpha}{2}+\mathrm{i}\mathbb{R}}
\frac{c_d(\alpha)c_d(d-\alpha-2w)}{c_d(d-2w)}
\mathcal{M}\varphi(w+\tfrac{\alpha}{2})\left|\cdot\right|^{-2w}\mathrm{d}w.
\end{align*}
Theorem (Riesz integral formula). Here is a Riesz integral formula mentioned in the Appendix of Landkof's book, in eq.~(1.6) of C.-Saff-Womersley, and in Remark 1 of Dyda-Kuznetsov-Kwaśnicki : if $d\geq1$ and $0<s<d$. Define, on $\mathbb{R}^d$,\[f:=(1-\left|\cdot\right|^2)_+^{\frac{s-d}{2}}.
\] Then, for all $x\in\mathbb{R}^d$ such that $0<|x|<1$,
\[
(\left|\cdot\right|^{-s}*f)(x)
=\pi^{\frac{d}{2}}\frac{\Gamma(\frac{d-s}{2})\Gamma(1-\frac{d-s}{2})}{\Gamma(\frac{d}{2})}
=\frac{\pi^{\frac{d}{2}+1}}{\Gamma(\frac{d}{2})\sin(\frac{d-s}{2}\pi)}.
\] The last equality simply comes from the Euler reflection formula $\Gamma(z)\Gamma(1-z)=\frac{\pi}{\sin(\pi z)}$.
Proof of the Riesz integral formula. The theorem is a special case of Corollary 4 in Dyda-Kuznetsov-Kwaśnicki, namely with $V\equiv1$, $l=0$, $\alpha=s-d$, $\delta=d$, $\rho = \sigma = -\frac{d-s}{2}$, $0<s<d-2$ (implies $\sigma>-1$). Let us give the proof extracted from there. With $\varphi(r):=(1-r)_+^{\frac{s-d}{2}}$, we have
\begin{equation}
\mathcal{M}\varphi(z)=\int_0^1r^{z-1}(1-r)^{\frac{s-d}{2}}\mathrm{d}r=\mathrm{Beta}(z,1-\tfrac{d-s}{2}),
\end{equation}and by the Lemma above,
\begin{align}
\mathcal{M}\psi(z)
&=\frac{\pi^{\frac{d}{2}}\Gamma(\frac{d-s}{2})\Gamma(1-\frac{d-s}{2})}
{\Gamma(\frac{s}{2})}
\frac{\Gamma(z)\Gamma(\frac{s}{2}-z)}{\Gamma(\frac{d}{2}-z)\Gamma(z+1)}.
\end{align} Now, if the vertical line $\lambda+\mathrm{i}\mathbb{R}$ separates the poles of $z\mapsto\Gamma(z)$ and of $z\mapsto\Gamma(\frac{s}{2}-z)$, then
\begin{align}
\frac{1}{2\pi\mathrm{i}}\int_{\lambda+\mathrm{i}\mathbb{R}}
\frac{\Gamma(z)\Gamma(\frac{s}{2}-z)}{\Gamma(\frac{d}{2}-z)\Gamma(z+1)}
x^{-z}\mathrm{d}z
&=\sum_{k=0}^\infty\mathrm{Residue}_{z=-k}\Bigr(\frac{\Gamma(z)\Gamma(\frac{s}{2}-z)}{\Gamma(\frac{d}{2}-z)\Gamma(z+1)}x^{-z}\Bigr)\\
&=\sum_{k=0}^\infty\frac{\Gamma(\frac{s}{2}+k)}{\Gamma(\frac{d}{2}+k)\Gamma(-k+1)}x^{k}\mathrm{Residue}_{z=-k}(\Gamma(z))\\
&=\sum_{k=0}^\infty\frac{\Gamma(\frac{s}{2}+k)}{\Gamma(\frac{d}{2}+k)\Gamma(-k+1)}\frac{(-x)^{k}}{k!} =\frac{\Gamma(\frac{s}{2})}{\Gamma(\frac{d}{2})}.
\end{align}
Recall that the $\Gamma$ function has no zeros, indeed a zero leads via $\Gamma(z)=(z-1)\Gamma(z-1)$ to infinitely many zeros to the left, and then via $\Gamma(z)\Gamma(1-z)=\frac{\pi}{\sin(\pi z)}$ to infinitely many poles to the right, which contradicts the analycity of $\Gamma$ on $\Re z>0$.
Recall also that the $\Gamma$ function is meromorphic on the complex plane, its poles are the non-positive integers, and are simple. Moreover, using $(z+n)\Gamma(z)=\frac{\Gamma(z+n+1)}{z(z+1)\cdots(z+n-1)}$ we get $$\mathrm{Residue}_{z=-n}(\Gamma(z)):=\lim_{z\to-n}(z-(-n))\Gamma(z)=\frac{(-1)^n}{n!}.$$
Meijer G-functions. A key point of the proof above is the computation of the inverse Mellin transform of a certain ratio of products of Gamma functions (Mellin transfrom of $\psi$). This is actually the definition of Meijer G-functions. If for example $$f(x):=(1-|x|^2)_+^\sigma\ {}_2F_1(a,b;c;1-|x|^2)=\varphi(|x|^2)$$ where $\varphi(r)=(1-r)_+^\sigma\ {}_2F_1(a,b;c;r)$, then it is possible, by using the same method as above, to express $\varphi$ as a Meijer G-function, and to deduce that the Riesz potential of $f$ on the unit ball is equal to another Meijer G-function, which reduces to a hypergeometric function in certain cases. This is explored in Dyda-Kuznetsov-Kwaśnicki and references therein.
Goody: Mellin transform of Gauss hypergeometric function. Recall the definition $${}_2F_1(a,b;c;z):=\sum_{n=0}^\infty\frac{(a)_n(b)_n}{(c)_n}\frac{z^n}{n!}$$ where $(a)_n:=a(a+1)\cdots(a+n-1)$ if $n\geq1$ and $(a)_0:=1$, in other words $(a)_n=\Gamma(a+n)/\Gamma(a)$. Now, if $f(x):={}_2F_1(a,b;c;-x)$ then \[\mathcal{M}f(z)
=\frac{\mathrm{Beta}(z,a-z)\mathrm{Beta}(z,b-z)}{\mathrm{Beta}(z,c-z)}
=\frac{\Gamma(c)}{\Gamma(a)\Gamma(b)}\frac{\Gamma(z)\Gamma(a-z)\Gamma(b-z)}{\Gamma(c-z)}.\] To see it, we can start from the Euler integral formula (which can be proved by a series expansion of $(1+xt)^{-b}$ using the Newton binomial series $(1-z)^{-\alpha}=\sum_{n=0}^\infty(\alpha)_n\frac{z^n}{n!}$) : \[{}_2F_1(a,b;c;-x) =\frac{\Gamma(c)}{\Gamma(a)\Gamma(c-a)} \int_0^1t^{a-1}(1-t)^{c-a-1}(1+xt)^{-b}\mathrm{d}t. \] This mixture representation gives, by the Fubini theorem, with $g_{t,b}(x):=(1+xt)^{-b}$,
\[
\mathcal{M}f(z)
=\frac{\Gamma(c)}{\Gamma(a)\Gamma(c-a)}
\int_0^1t^{a-1}(1-t)^{c-a-1}\mathcal{M}g_{t,b}(z)\mathrm{d}t
\quad\text{where}.
\] But by using the change of variable $y=(1+xt)^{-1}$ we get
\[
\mathcal{M}g_{t,b}(z)
=\int_0^\infty x^{z-1}g_{t,b}(x)\mathrm{d}x
=t^{-z}\int_0^1(1-y)^{z-1}y^{b-1-z}\mathrm{d}y
=t^{-z}\mathrm{Beta}(z,b-z),
\] It remains to note that $\int_0^1t^{a-1}(1-t)^{c-a-1}t^{-z}\mathrm{d}t=\mathrm{Beta}(c-a,a-z)$ and \[
\frac{\Gamma(c)}{\Gamma(a)\Gamma(c-a)}
\mathrm{Beta}(z,b-z)
\mathrm{Beta}(c-a,a-z)
=\frac{\Gamma(c)}{\Gamma(a)\Gamma(b)}\frac{\Gamma(z)\Gamma(a-z)\Gamma(b-z)}{\Gamma(c-z)}.
\] Alternatively, we could compute the inverse Mellin transform by using the residue formula.
Motivation. The original proof by Riesz of the integral formula, largely geometric, is also given in the Appendix of Landkof's book and of C.-Saff-Womersley. We were happy to locate a relatively short analytic proof, due to Dyda-Kuznetsov-Kwaśnicki, which is the subject of this post.
Further reading.
- On this blog
Unexpected phenomena for equilibrium measures
June 27, 2022 - D. Chafaï, E. B. Saff, and R. S. Womersley
Solution of a Riesz equilibrium problem and integral identities for special functions
J. Math. Anal. Appl. 515(1):29, 2022. Id/No 126367 - B. Davies
Integral transforms and their applications
Texts Appl. Math. 41. New York, NY: Springer. 3rd ed. edition, 2002 - B. Dyda, A. Kuznetsov, and M. Kwaśnicki
Fractional Laplace operator and Meijer G-function
Constr. Approx. 45(3):427–448, 2017 - N. S. Landkof, translated from the Russian by A. P. Doohovskoy
Foundations of modern potential theory
Grundlehren Math. Wiss. 180. Springer, Cham, 1972 - E. M. Stein
Singular integrals and differentiability properties of functions
Princeton Math. Ser. 30, Princeton University Press, Princeton, NJ, 1970 - M. Riesz
Sur certaines inégalités dans la théorie des fonctions avec quelques remarques sur les géometries non-euclidiennes
Kungl. Fysiogr. Sällsk. Lund Förh. 1, 21., 1931 - M. Riesz
Intégrales de Riemann-Liouville et potentiels
Acta Litt. Sci. Szeged, 9:1-42, 1938